Abstract
Transmembrane proteins comprise ~30% of the mammalian proteome, mediating metabolism, signalling, transport and many other functions required for cellular life. The microenvironment of integral membrane proteins (IMPs) is intrinsically different from that of cytoplasmic proteins, with IMPs solvated by a compositionally and biophysically complex lipid matrix. These solvating lipids affect protein structure and function in a variety of ways, from stereospecific, high-affinity protein–lipid interactions to modulation by bulk membrane properties. Specific examples of functional modulation of IMPs by their solvating membranes have been reported for various transporters, channels and signal receptors; however, generalizable mechanistic principles governing IMP regulation by lipid environments are neither widely appreciated nor completely understood. Here, we review recent insights into the inter-relationships between complex lipidomes of mammalian membranes, the membrane physicochemical properties resulting from such lipid collectives, and the regulation of IMPs by either or both. The recent proliferation of high-resolution methods to study such lipid–protein interactions has led to generalizable insights, which we synthesize into a general framework termed the ‘functional paralipidome’ to understand the mutual regulation between membrane proteins and their surrounding lipid microenvironments.
This is a preview of subscription content,access via your institution
Access options
Access Nature and 54 other Nature Portfolio journals
Get Nature+, our best-value online-access subscription
$29.99 per month
cancel any time
Subscribe to this journal
Receive 12 print issues and online access
$209.00 per year
only $17.42 per issue
Rent or buy this article
Get just this article for as long as you need it
$39.95
Prices may be subject to local taxes which are calculated during checkout
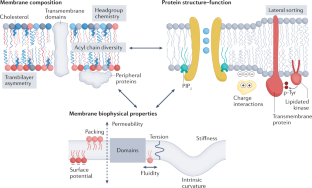
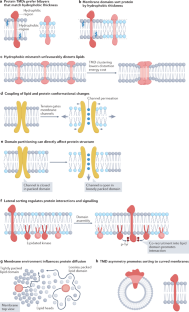
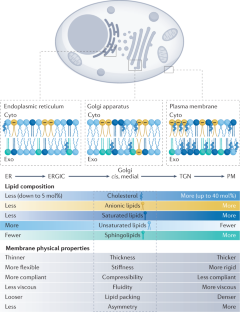
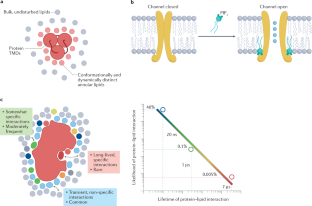
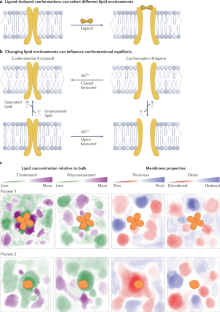
Change history
03 November 2022
A Correction to this paper has been published:https://doi.org/10.1038/s41580-022-00560-0
References
Krogh, A., Larsson, B., von Heijne, G. & Sonnhammer, E. L. Predicting transmembrane protein topology with a hidden Markov model: application to complete genomes.J. Mol. Biol.305, 567–580 (2001).
Overington, J. P., Al-Lazikani, B. & Hopkins, A. L. How many drug targets are there?Nat。牧师. Drug Discov.5, 993–996 (2006).
Payandeh, J. & Volgraf, M. Ligand binding at the protein-lipid interface: strategic considerations for drug design.Nat。牧师. Drug Discov.20, 710–722 (2021).
Harayama, T. & Riezman, H. Understanding the diversity of membrane lipid composition.Nat。牧师. Mol. Cell Biol.19, 281–296 (2018).
Capolupo, L. et al. Sphingolipids control dermal fibroblast heterogeneity.Science376, eabh1623 (2022).
Symons, J. L. et al. Lipidomic atlas of mammalian cell membranes reveals hierarchical variation induced by culture conditions, subcellular membranes, and cell lineages.Soft Matter17, 288–297 (2020).
Levental, K. R. et al. omega-3 polyunsaturated fatty acids direct differentiation of the membrane phenotype in mesenchymal stem cells to potentiate osteogenesis.Sci. Adv.3, eaao1193 (2017).Demonstrates that membrane lipidomes can be comprehensively remodelled by exogenous, dietary fatty acids and that these effects can modulate stem cell differentiation.
Han, X. Lipidomics for studying metabolism.Nat。牧师. Endocrinol.12, 668–679 (2016).
Eiriksson, F. F. et al. Lipidomic study of cell lines reveals differences between breast cancer subtypes.PLoS One15, e0231289 (2020).
Levental, K. R. et al. Lipidomic and biophysical homeostasis of mammalian membranes counteracts dietary lipid perturbations to maintain cellular fitness.Nat. Commun.11, 1339 (2020).
Contreras, F. X. et al. Molecular recognition of a single sphingolipid species by a protein’s transmembrane domain.Nature481, 525–529 (2012).Demonstration of the remarkable specificity of binding between a single-pass transmembrane domain and a particular sub-species of sphingomyelin.
Lemmon, M. A. Membrane recognition by phospholipid-binding domains.Nat。牧师. Mol. Cell. Biol.9, 99–111 (2008).
Moravcevic, K., Oxley, C. L. & Lemmon, M. A. Conditional peripheral membrane proteins: facing up to limited specificity.Structure20, 15–27 (2012).
Lee, A. G. How lipids affect the activities of integral membrane proteins.Biochim. Biophys. Acta1666, 62–87 (2004).
Barrera, N. P., Zhou, M. & Robinson, C. V. The role of lipids in defining membrane protein interactions: insights from mass spectrometry.Trends Cell Biol.23, 1–8 (2013).
Contreras, F. X., Ernst, A. M., Wieland, F. & Brugger, B. Specificity of intramembrane protein-lipid interactions.Cold Spring Harb. Perspect. Biol.3, a004705 (2011).
Corradi, V. et al. Emerging diversity in lipid-protein interactions.Chem. Rev.119, 5775–5848 (2019).
Sych, T., Levental, K. R. & Sezgin, E. Lipid-protein interactions in plasma membrane organization and function.Annu. Rev. Biophys.51, 135–156 (2022).
Ernst, M. & Robertson, J. L. The role of the membrane in transporter folding and activity.J. Mol. Biol.433, 167103 (2021).
Simunovic, M., Prevost, C., Callan-Jones, A. & Bassereau, P. Physical basis of some membrane shaping mechanisms.Philos. Trans. A Math. Phys. Eng. Sci.374, 20160034 (2016).
Phillips, R., Ursell, T., Wiggins, P. & Sens, P. Emerging roles for lipids in shaping membrane-protein function.Nature459, 379–385 (2009).
Heberle, F. A. et al. Direct label-free imaging of nanodomains in biomimetic and biological membranes by cryogenic electron microscopy.Proc. Natl Acad. Sci. USA117, 19943–19952 (2020).
Mitra, K., Ubarretxena-Belandia, I., Taguchi, T., Warren, G. & Engelman, D. M. Modulation of the bilayer thickness of exocytic pathway membranes by membrane proteins rather than cholesterol.Proc. Natl Acad. Sci. USA101, 4083–4088 (2004).
Cornell, C. E., Mileant, A., Thakkar, N., Lee, K. K. & Keller, S. L. Direct imaging of liquid domains in membranes by cryo-electron tomography.Proc. Natl Acad. Sci. USA117, 19713–19719 (2020).
Fischer, T. D., Dash, P. K., Liu, J. & Waxham, M. N. Morphology of mitochondria in spatially restricted axons revealed by cryo-electron tomography.PLoS Biol.16, e2006169 (2018).
Andersen, O. S. & Koeppe, R. E. 2nd Bilayer thickness and membrane protein function: an energetic perspective.Annu. Rev. Biophys. Biomol. Struct.36, 107–130 (2007).
Falzone, M. E. et al. TMEM16 scramblases thin the membrane to enable lipid scrambling.Nat. Commun.13, 2604 (2022).
Kaiser, H.-J. et al. Lateral sorting in model membranes by cholesterol-mediated hydrophobic matching.Proc. Natl Acad. Sci. USA108, 16628–16633 (2011).
Chadda, R. et al. Membrane transporter dimerization driven by differential lipid solvation energetics of dissociated and associated states.eLife10, e63288 (2021).Unique biochemical approaches are combined with simulations to show that IMP oligomerization is mediated by the energetics of TMD solvation by lipids rather than by direct lipid binding.
Beaven, A. H. et al. Gramicidin A channel formation induces local lipid redistribution I: experiment and simulation.Biophys. J.112, 1185–1197 (2017).
Sharpe, H. J., Stevens, T. J. & Munro, S. A comprehensive comparison of transmembrane domains reveals organelle-specific properties.Cell142, 158–169 (2010).
Diaz-Rohrer, B., Levental, K. R. & Levental, I. Rafting through traffic: membrane domains in cellular logistics.Biochim. Biophys. Acta Biomembr.1838, 3003–3013 (2014).
Prasad, R., Sliwa-Gonzalez, A. & Barral, Y. Mapping bilayer thickness in the ER membrane.Sci. Adv.6, aba5130 (2020).
Zhemkov, V. et al. The role of sigma 1 receptor in organization of endoplasmic reticulum signaling microdomains.eLife10, e65192 (2021).
King, C., Sengupta, P., Seo, A. Y. & Lippincott-Schwartz, J. ER membranes exhibit phase behavior at sites of organelle contact.Proc. Natl Acad. Sci. USA117, 7225–7235 (2020).
Cornelius, F. Modulation of Na,K-ATPase and Na-ATPase activity by phospholipids and cholesterol. I. Steady-state kinetics.Biochemistry40, 8842–8851 (2001).
Lee, A. G. Lipid-protein interactions in biological membranes: a structural perspective.Biochim. Biophys. Acta1612, 1–40 (2003).
Botelho, A. V., Huber, T., Sakmar, T. P. & Brown, M. F. Curvature and hydrophobic forces drive oligomerization and modulate activity of rhodopsin in membranes.Biophys. J.91, 4464–4477 (2006).
Jensen, M. O. & Mouritsen, O. G. Lipids do influence protein function — the hydrophobic matching hypothesis revisited.Biochim. Biophys. Acta Biomembr.1666, 205–226 (2004).A detailed discussion of experimental observations documenting how proteins perturb their lipid environments and, vice versa, how membrane properties like hydrophobic mismatch and curvature stress affect protein function.
Cybulski, L. E. et al. Activation of the bacterial thermosensor DesK involves a serine zipper dimerization motif that is modulated by bilayer thickness.Proc. Natl Acad. Sci. USA112, 6353–6358 (2015).
Simunovic, M., Evergren, E., Callan-Jones, A. & Bassereau, P. Curving cells inside and out: roles of BAR domain proteins in membrane shaping and its cellular implications.Annu. Rev. Cell Dev. Biol.35, 111–129 (2019).
Cui, H., Lyman, E. & Voth, G. A. Mechanism of membrane curvature sensing by amphipathic helix containing proteins.Biophys. J.100, 1271–1279 (2011).
Aimon, S. et al. Membrane shape modulates transmembrane protein distribution.Dev. Cell28, 212–218 (2014).
Shurer, C. R. et al. Physical principles of membrane shape regulation by the glycocalyx.Cell177, 1757–1770.e21 (2019).Protein engineering and scanning electron microscopy reveal remarkable morphological transformations of living cell membranes induced by glycosylated IMPs.
Stachowiak, J. C., Hayden, C. C. & Sasaki, D. Y. Steric confinement of proteins on lipid membranes can drive curvature and tubulation.Proc. Natl Acad. Sci. USA107, 7781–7786 (2010).
Ernst, A. M. et al. S-palmitoylation sorts membrane cargo for anterograde transport in the Golgi.Dev. Cell47, 479–493.e7 (2018).
Budin, I. et al. Viscous control of cellular respiration by membrane lipid composition.Science362, 1186–1189 (2018).巧妙的结合遗传学、生物物理学和垫子上hematical models to explain how membrane viscosity regulates cell growth via diffusion of electron carriers in the respiratory chain.
Sinensky, M. Homeoviscous adaptation-a homeostatic process that regulates the viscosity of membrane lipids in Escherichia coli.Proc. Natl Acad. Sci. USA71, 522–525 (1974).
Ernst, R., Ejsing, C. S. & Antonny, B. Homeoviscous adaptation and the regulation of membrane lipids.J. Mol. Biol.428, 4776–4791 (2016).
Martiniere, A. et al. Homeostasis of plasma membrane viscosity in fluctuating temperatures.N. Phytol.192, 328–337 (2011).
Behan-Martin, M. K., Jones, G. R., Bowler, K. & Cossins, A. R. A near perfect temperature adaptation of bilayer order in vertebrate brain membranes.Biochim. Biophys. Acta1151, 216–222 (1993).
Ruiz, M. et al. Membrane fluidity is regulated by the C. elegans transmembrane protein FLD-1 and its human homologs TLCD1/2.eLife7, 40686 (2018).
Covino, R. et al. A eukaryotic sensor for membrane lipid saturation.Mol. Cell63, 49–59 (2016).
Ballweg, S. et al. Regulation of lipid saturation without sensing membrane fluidity.Nat. Commun.11, 756 (2020).
Radanovic, T., Reinhard, J., Ballweg, S., Pesek, K. & Ernst, R. An emerging group of membrane property sensors controls the physical state of organellar membranes to maintain their identity.BioEssays40, e1700250 (2018).
Halbleib, K. et al. Activation of the unfolded protein response by lipid bilayer stress.Mol. Cell67, 673–684.e8 (2017).Demonstration that proteins can sense membrane properties by compressing the bilayer to signal aberrant lipid compositions during cell stress.
Cox, C. D., Bavi, N. & Martinac, B. Bacterial mechanosensors.Annu. Rev. Physiol.80, 71–93 (2018).
Reddy, B., Bavi, N., Lu, A., Park, Y. & Perozo, E. Molecular basis of force-from-lipids gating in the mechanosensitive channel MscS.eLife8, e50486 (2019).
Coste, B. et al. Piezo1 and Piezo2 are essential components of distinct mechanically activated cation channels.Science330, 55–60 (2010).
Lin, Y. C. et al. Force-induced conformational changes in PIEZO1.Nature573, 230–234 (2019).
Brohawn, S. G., Su, Z. & MacKinnon, R. Mechanosensitivity is mediated directly by the lipid membrane in TRAAK and TREK1 K+channels.Proc. Natl Acad. Sci. USA111, 3614–3619 (2014).
Bavi, N. et al. The conformational cycle of prestin underlies outer-hair cell electromotility.Nature600, 553–558 (2021).
Ge, J. et al. Molecular mechanism of prestin electromotive signal amplification.Cell184, 4669–4679 (2021).
Dallos, P. et al. Prestin-based outer hair cell motility is necessary for mammalian cochlear amplification.Neuron58, 333–339 (2008).
Sezgin, E., Levental, I., Mayor, S. & Eggeling, C. The mystery of membrane organization: composition, regulation and roles of lipid rafts.Nat。牧师. Mol. Cell Biol.18, 361–374 (2017).
Lingwood, D. & Simons, K. Lipid rafts as a membrane-organizing principle.Science327, 46–50 (2010).
Veatch, S. L. & Keller, S. L. Organization in lipid membranes containing cholesterol.Phys. Rev. Lett.89, 268101 (2002).
Veatch, S. L. & Keller, S. L. Seeing spots: complex phase behavior in simple membranes.Biochim. Biophys. Acta1746, 172–185 (2005).
Sodt, A. J., Sandar, M. L., Gawrisch, K., Pastor, R. W. & Lyman, E. The molecular structure of the liquid-ordered phase of lipid bilayers.J. Am. Chem. Soc.136, 725–732 (2014).
Sodt, A. J., Pastor, R. W. & Lyman, E. Hexagonal substructure and hydrogen bonding in liquid-ordered phases containing palmitoyl sphingomyelin.Biophys. J.109, 948–955 (2015).
Baumgart, T. et al. Large-scale fluid/fluid phase separation of proteins and lipids in giant plasma membrane vesicles.Proc. Natl Acad. Sci. USA104, 3165–3170 (2007).
Lingwood, D., Ries, J., Schwille, P. & Simons, K. Plasma membranes are poised for activation of raft phase coalescence at physiological temperature.Proc. Natl Acad. Sci. USA105, 10005–10010 (2008).
Levental, K. R. & Levental, I. Giant plasma membrane vesicles: models for understanding membrane organization.Curr. Top. Membr.75, 25–57 (2015).
Veatch, S. L. et al. Critical fluctuations in plasma membrane vesicles.ACS Chem. Biol.3, 287–293 (2008).
Levental, I. et al. Cholesterol-dependent phase separation in cell-derived giant plasma-membrane vesicles.Biochem. J.424, 163–167 (2009).
Levental, K. R. et al. Polyunsaturated lipids regulate membrane domain stability by tuning membrane order.Biophys. J.110, 1800–1810 (2016).
Toulmay, A. & Prinz, W. A. Direct imaging reveals stable, micrometer-scale lipid domains that segregate proteins in live cells.J. Cell Biol.202, 35–44 (2013).
Rayermann, S. P., Rayermann, G. E., Cornell, C. E., Merz, A. J. & Keller, S. L. Hallmarks of reversible separation of living, unperturbed cell membranes into two liquid phases.Biophys. J.113, 2425–2432 (2017).
Raghupathy, R. et al. Transbilayer lipid interactions mediate nanoclustering of lipid-anchored proteins.Cell161, 581 - 594(2015)。
Owen, D. M., Williamson, D. J., Magenau, A. & Gaus, K. Sub-resolution lipid domains exist in the plasma membrane and regulate protein diffusion and distribution.Nat. Commun.3, 1256 (2012).
Sanchez, S. A., Tricerri, M. A. & Gratton, E. Laurdan generalized polarization fluctuations measures membrane packing micro-heterogeneity in vivo.Proc. Natl Acad. Sci. USA109, 7314–7319 (2012).
Kinoshita, M. et al. Raft-based sphingomyelin interactions revealed by new fluorescent sphingomyelin analogs.J. Cell Biol.216, 1183–1204 (2017).
Komura, N. et al. Raft-based interactions of gangliosides with a GPI-anchored receptor.Nat. Chem. Biol.12, 402–410 (2016).
Stone, M. B., Shelby, S. A., Nunez, M. F., Wisser, K. & Veatch, S. L. Protein sorting by lipid phase-like domains supports emergent signaling function in B lymphocyte plasma membranes.eLife6, e19891 (2017).
Eggeling, C. et al. Direct observation of the nanoscale dynamics of membrane lipids in a living cell.Nature457, 1159–1162 (2009).
Levental, I., Levental, K. R. & Heberle, F. A. Lipid rafts: controversies resolved, mysteries remain.Trends Cell Biol.30, 341–353 (2020).
Sezgin, E. et al. Elucidating membrane structure and protein behavior using giant plasma membrane vesicles.Nat. Protoc.7, 1042–1051 (2012).
Levental Diaz-Rohrer, B, B。,西蒙斯,k和k·RLevental, I. Membrane raft association is a determinant of plasma membrane localization.Proc. Natl Acad. Sci. USA111, 8500–8505 (2014).
Lorent, j . h . et al。结构性因素和functional consequences of protein affinity for membrane rafts.Nat. Commun.8, 1219 (2017).
Levental, I., Lingwood, D., Grzybek, M., Coskun, U. & Simons, K. Palmitoylation regulates raft affinity for the majority of integral raft proteins.Proc. Natl Acad. Sci. USA107, 22050–22054 (2010).
Levental, I., Grzybek, M. & Simons, K. Greasing their way: lipid modifications determine protein association with membrane rafts.Biochemistry49, 6305–6316 (2010).
Castello-Serrano, I., Lorent, J. H., Ippolito, R., Levental, K. R. & Levental, I. Myelin-associated MAL and PLP Are unusual among multipass transmembrane proteins in preferring ordered membrane domains.J. Phys. Chem. B124, 5930–5939 (2020).
Sengupta, P. et al. A lipid-based partitioning mechanism for selective incorporation of proteins into membranes of HIV particles.Nat. Cell Biol.21, 452–461 (2019).
Ono, A. & Freed, E. O. Plasma membrane rafts play a critical role in HIV-1 assembly and release.Proc. Natl Acad. Sci. USA98, 13925–13930 (2001).
Mitchell, D. C., Straume, M., Miller, J. L. & Litman, B. J. Modulation of metarhodopsin formation by cholesterol-induced ordering of bilayer lipids.Biochemistry29, 9143–9149 (1990).
Huang, S. K. et al. Allosteric modulation of the adenosine A2A receptor by cholesterol.Elife11, e73901 (2021).
Tikku, S. et al. Relationship between Kir2.1/Kir2.3 activity and their distributions between cholesterol-rich and cholesterol-poor membrane domains.Am. J. Physiol. Cell Physiol.293, C440–C450 (2007).
Murata, M. et al. VIP21/caveolin is a cholesterol-binding protein.Proc. Natl Acad. Sci. USA92, 10339–10343 (1995).
Parton, R. G. & Simons, K. The multiple faces of caveolae.Nat。牧师. Mol. Cell Biol.8, 185–194 (2007).
Parton, R. G., Kozlov, M. M. & Ariotti, N. Caveolae and lipid sorting: shaping the cellular response to stress.J. Cell Biol.219, e201905071 (2020).
Ortegren, U. et al. Lipids and glycosphingolipids in caveolae and surrounding plasma membrane of primary rat adipocytes.Eur. J. Biochem.271, 2028–2036 (2004).
Rothberg, K. G. et al. Caveolin, a protein component of caveolae membrane coats.Cell68, 673–682 (1992).
Epand, R. M., Sayer, B. G. & Epand, R. F. Caveolin scaffolding region and cholesterol-rich domains in membranes.J. Mol. Biol.345, 339–350 (2005).
Sinha, B. et al. Cells respond to mechanical stress by rapid disassembly of caveolae.Cell144, 402–413 (2011).
Marsh, D. Protein modulation of lipids, and vice-versa, in membranes.Biochim. Biophys. Acta1778, 1545–1575 (2008).
Gonen, T. et al. Lipid-protein interactions in double-layered two-dimensional AQP0 crystals.Nature438, 633–638 (2005).Ground-breaking study using electron diffraction from two-dimension crystals of a membrane protein, AQP0, providing direct evidence for lipid–protein interaction and the water conduction mechanism.
Jost, P. C., Griffith, O. H., Capaldi, R. A. & Vanderkooi, G. Evidence for boundary lipid in membranes.Proc. Natl Acad. Sci. USA70, 480–484 (1973).
Brotherus, j . r . et al。Lipid-protein多个本ding equilibria in membranes.Biochemistry20, 5261–5267 (1981).
阅读l . I。Brophy, p . j . &沼泽,d .交换rates at the lipid-protein interface of myelin proteolipid protein studied by spin-label electron spin resonance.Biochemistry27, 46–52 (1988).
Marius, P., Alvis, S. J., East, J. M. & Lee, A. G. The interfacial lipid binding site on the potassium channel KcsA is specific for anionic phospholipids.Biophys. J.89, 4081–4089 (2005).
McAuley, K. E. et al. Structural details of an interaction between cardiolipin and an integral membrane protein.Proc. Natl Acad. Sci. USA96, 14706–14711 (1999).
Belrhali, H. et al. Protein, lipid and water organization in bacteriorhodopsin crystals: a molecular view of the purple membrane at 1.9 A resolution.Structure7, 909–917 (1999).
Zhou, Y., Morais-Cabral, J. H., Kaufman, A. & MacKinnon, R. Chemistry of ion coordination and hydration revealed by a K+channel-Fab complex at 2.0 A resolution.Nature414, 43–48 (2001).
Duncan, A. L., Song, W. & Sansom, M. S. P. Lipid-dependent regulation of ion channels and G protein-coupled receptors: insights from structures and simulations.Annu. Rev. Pharmacol. Toxicol.60, 31–50 (2020).
Hansen, S. B., Tao, X. & MacKinnon, R. Structural basis of PIP2 activation of the classical inward rectifier K+channel Kir2.2.Nature477, 495–498 (2011).
McLaughlin, S., Wang, J., Gambhir, A. & Murray, D. PIP(2) and proteins: interactions, organization, and information flow.Annu. Rev. Biophys. Biomol. Struct.31, 151–175 (2002).
Rosenhouse-Dantsker, A., Noskov, S., Durdagi, S., Logothetis, D. E. & Levitan, I. Identification of novel cholesterol-binding regions in Kir2 channels.J. Biol. Chem.288, 31154–31164 (2013).
Romanenko, V. G. et al. Cholesterol sensitivity and lipid raft targeting of Kir2.1 channels.Biophys. J.87, 3850–3861 (2004).
Duncan, A. L., Corey, R. A. & Sansom, M. S. P. Defining how multiple lipid species interact with inward rectifier potassium (Kir2) channels.Proc. Natl Acad. Sci. USA117, 7803–7813 (2020).
Zaydman, M. A. et al. Kv7.1 ion channels require a lipid to couple voltage sensing to pore opening.Proc. Natl Acad. Sci. USA110, 13180–13185 (2013).
Hilgemann, D. W. & Ball, R. Regulation of cardiac Na+,Ca2+exchange and KATP potassium channels by PIP2.Science273, 956–959 (1996).
Hedger, G. et al. Lipid-loving ANTs: molecular simulations of cardiolipin interactions and the organization of the adenine nucleotide translocase in model mitochondrial membranes.Biochemistry55, 6238–6249 (2016).
Hite, R. K., Butterwick, J. A. & MacKinnon, R. Phosphatidic acid modulation of Kv channel voltage sensor function.eLife3, e04366 (2014).
Zocher, M., Zhang, C., Rasmussen, S. G., Kobilka, B. K. & Muller, D. J. Cholesterol increases kinetic, energetic, and mechanical stability of the human beta2-adrenergic receptor.Proc. Natl Acad. Sci. USA109, E3463–E3472 (2012).
Cherezov, V. et al. High-resolution crystal structure of an engineered human beta2-adrenergic G protein-coupled receptor.Science318, 1258–1265 (2007).
Liu, W. et al. Structural basis for allosteric regulation of GPCRs by sodium ions.Science337, 232–236 (2012).
Guixa-Gonzalez, R. et al. Membrane cholesterol access into a G-protein-coupled receptor.Nat. Commun.8, 14505 (2017).
Manna, M. et al. Mechanism of allosteric regulation of beta2-adrenergic receptor by cholesterol.eLife5, e18432 (2016).
Rouviere, E., Arnarez, C., Yang, L. & Lyman, E. Identification of two new cholesterol interaction sites on the A2A adenosine receptor.Biophys. J.113, 2415–2424 (2017).
Lee, J. Y. & Lyman, E. Predictions for cholesterol interaction sites on the A2A adenosine receptor.J. Am. Chem. Soc.134, 16512–16515 (2012).
Nelson, L. D., Johnson, A. E. & London, E. How interaction of perfringolysin O with membranes is controlled by sterol structure, lipid structure, and physiological low pH: insights into the origin of perfringolysin O-lipid raft interaction.J. Biol. Chem.283, 4632–4642 (2008).
D’Avanzo, N., Hyrc, K., Enkvetchakul, D., Covey, D. F. & Nichols, C. G. Enantioselective protein-sterol interactions mediate regulation of both prokaryotic and eukaryotic inward rectifier K+channels by cholesterol.PLoS One6, e19393 (2011).
Gutorov, R. et al. Modulation of transient receptor potential C channel activity by cholesterol.Front. Pharmacol.10, 1487 (2019).
Elkins, M. R. et al. Cholesterol-binding site of the influenza M2 protein in lipid bilayers from solid-state NMR.Proc. Natl Acad. Sci. USA114, 12946–12951 (2017).
Barrett, P. J. et al. The amyloid precursor protein has a flexible transmembrane domain and binds cholesterol.Science336, 1168–1171 (2012).
Marlow, B., Kuenze, G., Li, B., Sanders, C. R. & Meiler, J. Structural determinants of cholesterol recognition in helical integral membrane proteins.Biophys. J.120, 1592–1604 (2021).
Romer, W. et al. Shiga toxin induces tubular membrane invaginations for its uptake into cells.Nature450, 670–675 (2007).
Flores, A. et al. Gangliosides interact with synaptotagmin to form the high-affinity receptor complex for botulinum neurotoxin B.Proc. Natl Acad. Sci. USA116, 18098–18108 (2019).
Ewers, H. et al. GM1 structure determines SV40-induced membrane invagination and infection.Nat. Cell Biol.12, 11–18 (2010).
Coskun, U., Grzybek, M., Drechsel, D. & Simons, K. Regulation of human EGF receptor by lipids.Proc. Natl Acad. Sci. USA108, 9044–9048 (2010).
Song, W., Yen, H. Y., Robinson, C. V. & Sansom, M. S. P. State-dependent lipid interactions with the A2a receptor revealed by MD simulations using in vivo-mimetic membranes.Structure27, 392–403.e3 (2019).
Laganowsky, A. et al. Membrane proteins bind lipids selectively to modulate their structure and function.Nature510, 172–175 (2014).Native mass spectrometry used to demonstrate that lipids can remain bound to proteins through the extraction and ionization process and be identified directly by their molecular mass.
Cong, X. et al. Determining membrane protein-lipid binding thermodynamics using native mass spectrometry.J. Am. Chem. Soc.138, 4346–4349 (2016).
Patrick, J. W. et al. Allostery revealed within lipid binding events to membrane proteins.Proc. Natl Acad. Sci. USA115, 2976–2981 (2018).
Gupta, K. et al. The role of interfacial lipids in stabilizing membrane protein oligomers.Nature541, 421–424 (2017).
Yang, L. & Lyman, E. Local enrichment of unsaturated chains around the A2A adenosine receptor.Biochemistry58, 4096–4105 (2019).
Tanford, C. Extension of the theory of linked functions to incorporate the effects of protein hydration.J. Mol. Biol.39, 539–544 (1969).
Salari, R., Joseph, T., Lohia, R., Henin, J. & Brannigan, G. A streamlined, general approach for computing ligand binding free energies and its application to GPCR-bound cholesterol.J. Chem. Theory Comput.14, 6560–6573 (2018).
Corey, R. A., Stansfeld, P. J. & Sansom, M. S. P. The energetics of protein-lipid interactions as viewed by molecular simulations.Biochem. Soc. Trans.48, 25–37 (2020).
Salas-Estrada, L. A., Leioatts, N., Romo, T. D. & Grossfield, A. Lipids alter rhodopsin function via ligand-like and solvent-like interactions.Biophys. J.114, 355–367 (2018).
Leonard, A. N. & Lyman, E. Activation of G-protein-coupled receptors is thermodynamically linked to lipid solvation.Biophys. J.120, 1777–1787 (2021).Conceptual underpinning of the functional paralipidome model, demonstrating via computational and theoretical analysis, how lipid nano-environments could influence protein conformational equilibria.
Huber, T., Botelho, A. V., Beyer, K. & Brown, M. F. Membrane model for the G-protein-coupled receptor rhodopsin: hydrophobic interface and dynamical structure.Biophys. J.86, 2078–2100 (2004).
van ‘t Klooster, J. S. et al. Periprotein lipidomes of Saccharomyces cerevisiae provide a flexible environment for conformational changes of membrane proteins.eLife9, e57003 (2020).Demonstration of the remarkable specificity of binding between a single-pass transmembrane domain and a particular sub-species of sphingomyelin.
Gupta, K. et al. Identifying key membrane protein lipid interactions using mass spectrometry.Nat. Protoc.13, 1106–1120 (2018).
Corradi, V. et al. Lipid-protein interactions are unique fingerprints for membrane proteins.ACS Cent. Sci.4, 709–717 (2018).
Denisov, I. G. & Sligar, S. G. Nanodiscs for structural and functional studies of membrane proteins.Nat. Struct. Mol. Biol.23, 481–486 (2016).
Dorr, J. M. et al. Detergent-free isolation, characterization, and functional reconstitution of a tetrameric K+ channel: the power of native nanodiscs.Proc. Natl Acad. Sci. USA111, 18607–18612 (2014).
Flores, J. A. et al. Connexin-46/50 in a dynamic lipid environment resolved by CryoEM at 1.9 A.Nat. Commun.11, 4331 (2020).Ultra-high-resolution structures of native connexin channels, which span two bilayers, reveal novel details of IMP–membrane interactions, including remarkable ordering of outer leaflet lipids.
Cuevas Arenas, R. et al. Fast collisional lipid transfer among polymer-bounded nanodiscs.Sci. Rep.7, 45875 (2017).
Stepien, P. et al. Complexity of seemingly simple lipid nanodiscs.Biochim. Biophys. Acta Biomembr.1862, 183420 (2020).
Javanainen, M. et al. Reduced level of docosahexaenoic acid shifts GPCR neuroreceptors to less ordered membrane regions.PLoS Comput. Biol.15, e1007033 (2019).
Sodt, A. J., Beaven, A. H., Andersen, O. S., Im, W. & Pastor, R. W. Gramicidin a channel formation induces local lipid redistribution II: a 3D continuum elastic model.Biophys. J.112, 1198–1213 (2017).
Lundbaek, j ., Collingwood, s。Ingolfsson, H. I., Kapoor, R. & Andersen, O. S. Lipid bilayer regulation of membrane protein function: gramicidin channels as molecular force probes.j . r . Soc。接口7, 373–395 (2010).
McGraw, C., Yang, L., Levental, I., Lyman, E. & Robinson, A. S. Membrane cholesterol depletion reduces downstream signaling activity of the adenosine A2A receptor.Biochim. Biophys. Acta Biomembr.1861, 760–767 (2019).
Gutierrez, M. G., Mansfield, K. S. & Malmstadt, N. The functional activity of the human serotonin 5-HT1A receptor is controlled by lipid bilayer composition.Biophys. J.110, 2486–2495 (2016).
Kumar, G. A. et al. A molecular sensor for cholesterol in the human serotonin1A receptor.Sci. Adv.7, eabh2922 (2021).
Barbotin, A. et al. z-STED imaging and spectroscopy to investigate nanoscale membrane structure and dynamics.Biophys. J.118, 2448–2457 (2020).
Nadezhdin, K. D. et al. Structural mechanism of heat-induced opening of a temperature-sensitive TRP channel.Nat. Struct. Mol. Biol.28, 564–572 (2021).
Doktorova, M., Symons, J. L. & Levental, I. Structural and functional consequences of reversible lipid asymmetry in living membranes.Nat. Chem. Biol.16, 1321–1330 (2020).
Lorent, j . h . et al。Plasma membranes are asymmetric in lipid unsaturation, packing and protein shape.Nat. Chem. Biol.16, 644–652 (2020).Experiments and computational modelling detail the lipidomic and biophysical asymmetry of a mammalian plasma membrane, while bioinformatics shows that membrane asymmetry is reflected in asymmetry of protein TMDs, which is conserved throughout Eukaryota.
Tsuji, T. et al. Predominant localization of phosphatidylserine at the cytoplasmic leaflet of the ER, and its TMEM16K-dependent redistribution.Proc. Natl Acad. Sci. USA116, 13368–13373 (2019).
Malvezzi, M. et al. Ca2+-dependent phospholipid scrambling by a reconstituted TMEM16 ion channel.Nat. Commun.4, 2367 (2013).
van Meer, G., Voelker, D. R. & Feigenson, G. W. Membrane lipids: where they are and how they behave.Nat。牧师. Mol. Cell Biol.9, 112–124 (2008).
Akimzhanov, A. M. & Boehning, D. Rapid and transient palmitoylation of the tyrosine kinase Lck mediates Fas signaling.Proc. Natl Acad. Sci. USA112, 11876–11880 (2015).
Martin, B. R., Wang, C., Adibekian, A., Tully, S. E. & Cravatt, B. F. Global profiling of dynamic protein palmitoylation.Nat. Methods9, 84–89 (2011).
Martin, B. R. & Cravatt, B. F. Large-scale profiling of protein palmitoylation in mammalian cells.Nat. Methods6, 135–138 (2009).
Veatch, S. L., Gawrisch, K. & Keller, S. L. Closed-loop miscibility gap and quantitative tie-lines in ternary membranes containing diphytanoyl PC.Biophys. J.90, 4428–4436 (2006).
Feigenson, G. W. & Buboltz, J. T. Ternary phase diagram of dipalmitoyl-PC/dilauroyl-PC/cholesterol: nanoscopic domain formation driven by cholesterol.Biophys. J.80, 2775–2788 (2001).
Sezgin, E. et al. Partitioning, diffusion, and ligand binding of raft lipid analogs in model and cellular plasma membranes.Biochim. Biophys. Acta1818, 1777–1784 (2012).
Kaiser, H. J. et al. Order of lipid phases in model and plasma membranes.Proc. Natl Acad. Sci. USA106, 16645–16650 (2009).
Gerl, M. J. et al. Quantitative analysis of the lipidomes of the influenza virus envelope and MDCK cell apical membrane.J. Cell Biol.196, 213–221 (2012).
Sampaio, J. L. et al. Membrane lipidome of an epithelial cell line.Proc. Natl Acad. Sci. USA108, 1903–1907 (2011).
Franck, J. M., Chandrasekaran, S., Dzikovski, B., Dunnam, C. R. & Freed, J. H. Focus: two-dimensional electron-electron double resonance and molecular motions: the challenge of higher frequencies.J. Chem. Phys.142, 212302 (2015).
Kuerschner, L. et al. Polyene-lipids: a new tool to image lipids.Nat. Methods2, 39–45 (2005).
Ward, A. E., Ye, Y., Schuster, J. A., Wei, S. & Barrera, F. N. Single-molecule fluorescence vistas of how lipids regulate membrane proteins.Biochem. Soc. Trans.49, 1685–1694 (2021).
McIntosh, A. L. et al. Fluorescence techniques using dehydroergosterol to study cholesterol trafficking.Lipids43, 1185–1208 (2008).
Thiele, C., Hannah, M. J., Fahrenholz, F. & Huttner, W. B. Cholesterol binds to synaptophysin and is required for biogenesis of synaptic vesicles.Nat. Cell Biol.2, 42–49 (2000).
Hoglinger, D. et al. Trifunctional lipid probes for comprehensive studies of single lipid species in living cells.Proc. Natl Acad. Sci. USA114, 1566–1571 (2017).
Schuhmacher, M. et al. Live-cell lipid biochemistry reveals a role of diacylglycerol side-chain composition for cellular lipid dynamics and protein affinities.Proc. Natl Acad. Sci. USA117, 7729–7738 (2020).
Romero, L. O. et al. Dietary fatty acids fine-tune Piezo1 mechanical response.Nat. Commun.10, 1200 (2019).
Chakrapani, S., Cordero-Morales, J. F. & Perozo, E. A quantitative description of KcsA gating I: macroscopic currents.J. Gen. Physiol.130, 465–478 (2007).
Garten, M. et al. Whole-GUV patch-clamping.Proc. Natl Acad. Sci. USA114, 328–333 (2017).
Newport, T. D., Sansom, M. S. P. & Stansfeld, P. J. The MemProtMD database: a resource for membrane-embedded protein structures and their lipid interactions.Nucleic Acids Res.47, D390–D397 (2019).
Kimura, Y. et al. Surface of bacteriorhodopsin revealed by high-resolution electron crystallography.Nature389, 206–211 (1997).
Reichow, S. L. & Gonen, T. Lipid-protein interactions probed by electron crystallography.Curr. Opin. Struct. Biol.19, 560–565 (2009).
Han, X., Yang, K. & Gross, R. W. Multi-dimensional mass spectrometry-based shotgun lipidomics and novel strategies for lipidomic analyses.Mass. Spectrom. Rev.31, 134–178 (2012).
Laganowsky, A., Reading, E., Hopper, J. T. & Robinson, C. V. Mass spectrometry of intact membrane protein complexes.Nat. Protoc.8, 639–651 (2013).
Acknowledgements
Major acknowledgement goes to K. Levental for insightful feedback and for generating the beautiful and informative figures for the manuscript. We also acknowledge the members of the Levental and Lyman labs for critical reading and enlightening discussions that have polished the ideas in the manuscript. This work was supported by NIH/National Institute of General Medical Sciences (GM134949, GM124072, GM120351), the Volkswagen Foundation (grant 93091) and the Human Frontiers Science Program (RGP0059/2019).
Author information
Authors and Affiliations
Contributions
Both authors contributed equally to all aspects of the article.
Corresponding authors
Ethics declarations
Competing interests
The authors declare no competing interests.
Peer review
Peer review information
Nature Reviews Molecular Cell Biologythanks Richard Epand, Janice Robertson and Peter Tieleman for their contribution to the peer review of this work.
Additional information
Publisher’s note
关于文书期刊上施普林格自然保持中立isdictional claims in published maps and institutional affiliations.
Glossary
- Unfolded protein response
-
A conserved cellular response to various stresses to the protein folding and secretory systems; can also be induced by lipid perturbations.
- MscL and MscS families
-
Bacterial mechanosensitive channels that open in response to membrane tension, for example, induced by osmotic shock.
- PIP2
-
Phosphatidylinositol 4,5-bisphosphate, a highly charged and tightly regulated lipid type that can associate tightly with integral membrane proteins through both electrostatic and stereospecific interactions.
- Sphingolipids
-
Lipid with sphingoid backbones rather than glycerol. Lipids of this class (for example, sphingomyelin) are common components of eukaryote plasma membranes, interact well with sterols and bind strongly to some proteins.
- Native mass spectrometry
-
A mass spectrometric technique capable of measuring molecular weights of large macromolecules (that is, proteins and their complexes) without fragmentation.
- Shotgun lipidomics
-
A mass spectrometric technique for identifying and quantifying the lipid components of a complex sample (for example, cell membrane) without prior chromatographic separation.
- Nanodiscs
-
An experimental construct containing an integral membrane protein, lipids and a scaffold that solubilizes them. The scaffold can be a protein (MSP) or synthetic polymer (for example, styrene maleic acid).
- Gramicidin A
-
An antibiotic peptide that assembles to form transmembrane pores, dependent on its membrane environment.
Rights and permissions
Springer Nature or its licensor (e.g. a society or other partner) holds exclusive rights to this article under a publishing agreement with the author(s) or other rightsholder(s); author self-archiving of the accepted manuscript version of this article is solely governed by the terms of such publishing agreement and applicable law.
About this article
Cite this article
Levental, I., Lyman, E. Regulation of membrane protein structure and function by their lipid nano-environment.Nat Rev Mol Cell Biol24, 107–122 (2023). https://doi.org/10.1038/s41580-022-00524-4
Accepted:
Published:
Issue Date:
DOI:https://doi.org/10.1038/s41580-022-00524-4
This article is cited by
The lipotype hypothesis
Nature Reviews Molecular Cell Biology(2023)