Abstract
Folates are essential nutrients with important roles as cofactors in one-carbon transfer reactions, being heavily utilized in the synthesis of nucleic acids and the metabolism of amino acids during cell division1,2. Mammals lack de novo folate synthesis pathways and thus rely on folate uptake from the extracellular milieu3. The human reduced folate carrier (hRFC, also known as SLC19A1) is the major importer of folates into the cell1,3, as well as chemotherapeutic agents such as methotrexate4,5,6. As an anion exchanger, RFC couples the import of folates and antifolates to anion export across the cell membrane and it is a major determinant in methotrexate (antifolate) sensitivity, as genetic variants and its depletion result in drug resistance4,5,6,7,8. Despite its importance, the molecular basis of substrate specificity by hRFC remains unclear. Here we present cryo-electron microscopy structures of hRFC in the apo state and captured in complex with methotrexate. Combined with molecular dynamics simulations and functional experiments, our study uncovers key determinants of hRFC transport selectivity among folates and antifolate drugs while shedding light on important features of anion recognition by hRFC.
This is a preview of subscription content,access via your institution
Access options
Subscribe to Nature+
Get immediate online access to Nature and 55 other Nature journal
$29.99
monthly
Subscribe to Journal
Get full journal access for 1 year
$199.00
only $3.90 per issue
All prices are NET prices.
VAT will be added later in the checkout.
Tax calculation will be finalised during checkout.
Buy article
Get time limited or full article access on ReadCube.
$32.00
All prices are NET prices.
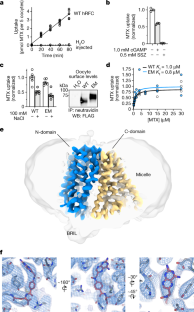
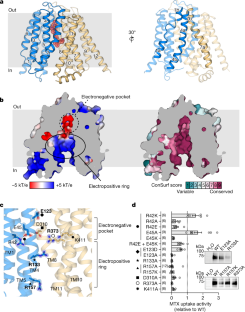
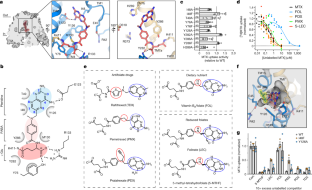
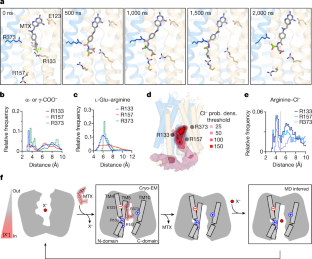
Data availability
Coordinates have been deposited in the Protein Data Bank with IDs7TX6(hRFCEM–MTX),7XT7(hRFCEM) and8DEP(apo hRFCEM). The cryo-EM maps have been deposited in the Electron Microscopy Data Bank with IDs EMD-26155(hRFCEM–MTX), EMD-26156(hRFCEM) and EMD-27394(apo hRFCEM).Source dataare provided with this paper.
References
Zheng, Y. & Cantley, L. C. Toward a better understanding of folate metabolism in health and disease.J. Exp. Med.216, 253–266 (2019).
Clare, C. E., Brassington, A. H., Kwong, W. Y. & Sinclair, K. D. One-carbon metabolism: linking nutritional biochemistry to epigenetic programming of long-term development.Annu. Rev. Anim. Biosci.7, 263–287 (2019).
Hou, Z. & Matherly, L. H. Biology of the major facilitative folate transporters SLC19A1 and SLC46A1.Curr. Top. Membr.73, 175–204 (2014).
Matherly, L. H., Hou, Z. & Deng, Y. Human reduced folate carrier: translation of basic biology to cancer etiology and therapy.癌症Metastasis Rev.26, 111 - 128(2007)。
O’Connor, C. et al. Folate transporter dynamics and therapy with classic and tumor-targeted antifolates.Sci. Rep.11, 6389 (2021).
Kanarek, N. et al. Histidine catabolism is a major determinant of methotrexate sensitivity.Nature559, 632–636 (2018).
Kobayashi, H., Takemura, Y. & Ohnuma, T. Variable expression of RFC1 in human leukemia cell lines resistant to antifolates.癌症Lett.124, 135–142 (1998).
Girardi, E. et al. A widespread role for SLC transmembrane transporters in resistance to cytotoxic drugs.Nat. Chem. Biol.16, 469–478 (2020).
Zhao, R. et al. Rescue of embryonic lethality in reduced folate carrier-deficient mice by maternal folic acid supplementation reveals early neonatal failure of hematopoietic organs.J. Biol. Chem.276, 10224–10228 (2001).
Svaton, M. et al. A homozygous deletion in the SLC19A1 gene as a cause of folate-dependent recurrent megaloblastic anemia.Blood135, 2427–2431 (2020).
Yang, R. et al. Sequence alterations in the reduced folate carrier are observed in osteosarcoma tumor samples.Clin. Cancer Res.9, 837–844 (2003).
Matherly, L. H. & Hou, Z. Structure and function of the reduced folate carrier a paradigm of a major facilitator superfamily mammalian nutrient transporter.Vitam. Horm.79, 145–184 (2008).
Yee, S. W. et al. SLC19A1 pharmacogenomics summary.Pharmacogenet. Genomics20, 708–715 (2010).
Guo, W. et al. Mechanisms of methotrexate resistance in osteosarcoma.Clin. Cancer Res.5, 621–627 (1999).
Luteijn, R. D. et al. SLC19A1 transports immunoreactive cyclic dinucleotides.Nature573, 434–438 (2019).
Ritchie, C., Cordova, A. F., Hess, G. T., Bassik, M. C. & Li, L. SLC19A1 is an importer of the immunotransmitter cGAMP.Mol. Cell75, 372 - 381。e5(2019)。
Alam, C., Hoque, M. T., Finnell, R. H., Goldman, I. D. & Bendayan, R. Regulation of reduced folate carrier (RFC) by vitamin D receptor at the blood-brain barrier.Mol. Pharm.14, 3848–3858 (2017).
Hou, Z., Ye, J., Haska, C. L. & Matherly, L. H. Transmembrane domains 4, 5, 7, 8, and 10 of the human reduced folate carrier are important structural or functional components of the transmembrane channel for folate substrates.J. Biol. Chem.281, 33588–33596 (2006).
Ganapathy, V., Smith, S. B. & Prasad, P. D. SLC19: the folate/thiamine transporter family.Pflugers Arch.447, 641–646 (2004).
Henderson, G. B. & Zevely, E. M. Anion exchange mechanism for transport of methotrexate in L1210 cells.Biochem. Biophys. Res. Commun.99, 163–169 (1981).
Zhao, R., Gao, F. & Goldman, I. D. Reduced folate carrier transports thiamine monophosphate: an alternative route for thiamine delivery into mammalian cells.Am. J. Physiol. Cell Physiol.282, C1512–C1517 (2002).
Goldman, I. D. The characteristics of the membrane transport of amethopterin and the naturally occurring folates.Ann. NY Acad. Sci.186, 400–422 (1971).
Zhao, R., Gao, F., Hanscom, M. & Goldman, I. D. A prominent low-pH methotrexate transport activity in human solid tumors: contribution to the preservation of methotrexate pharmacologic activity in HeLa cells lacking the reduced folate carrier.Clin. Cancer Res.10, 718–727 (2004).
Desmoulin, S. K., Hou, Z., Gangjee, A. & Matherly, L. H. The human proton-coupled folate transporter: biology and therapeutic applications to cancer.癌症Biol. Ther.13, 1355–1373 (2012).
Parker, J. L. et al. Structural basis of antifolate recognition and transport by PCFT.Nature595, 130–134 (2021).
Jansen, G. et al. Sulfasalazine is a potent inhibitor of the reduced folate carrier: implications for combination therapies with methotrexate in rheumatoid arthritis.Arthritis Rheum.50, 2130–2139 (2004).
Straub, M. S., Alvadia, C., Sawicka, M. & Dutzler, R. Cryo-EM structures of the caspase-activated protein XKR9 involved in apoptotic lipid scrambling.Elife10, e69800 (2021).
Chun, E. et al. Fusion partner toolchest for the stabilization and crystallization of G protein-coupled receptors.Structure20, 967–976 (2012).
Gao, X. et al. Mechanism of substrate recognition and transport by an amino acid antiporter.Nature463, 828–832 (2010).
Henderson, G. B. & Zevely, E. M. Affinity labeling of the 5-methyltetrahydrofolate/methotrexate transport protein of L1210 cells by treatment with an N-hydroxysuccinimide ester of [3H]methotrexate.J. Biol. Chem.259, 4558–4562 (1984).
Hou, Z., Stapels, S. E., Haska, C. L. & Matherly, L. H. Localization of a substrate binding domain of the human reduced folate carrier to transmembrane domain 11 by radioaffinity labeling and cysteine-substituted accessibility methods.J. Biol. Chem.280, 36206–36213 (2005).
Deng, Y. et al. Role of lysine 411 in substrate carboxyl group binding to the human reduced folate carrier, as determined by site-directed mutagenesis and affinity inhibition.Mol. Pharmacol.73, 1274–1281 (2008).
Liu, X. Y. & Matherly, L. H. Functional interactions between arginine-133 and aspartate-88 in the human reduced folate carrier: evidence for a charge-pair association.Biochem. J.358, 511–516 (2001).
Brigle, K. E., Spinella, M. J., Sierra, E. E. & Goldman, I. D. Characterization of a mutation in the reduced folate carrier in a transport defective L1210 murine leukemia cell line.J. Biol. Chem.270, 22974–22979 (1995).
Zhao, R., Sharina, I. G. & Goldman, I. D. Pattern of mutations that results in loss of reduced folate carrier function under antifolate selective pressure augmented by chemical mutagenesis.Mol. Pharmacol.56, 68–76 (1999).
Rothem, L. et al. Resistance to multiple novel antifolates is mediated via defective drug transport resulting from clustered mutations in the reduced folate carrier gene in human leukaemia cell lines.Biochem. J.367, 741–750 (2002).
Tse, A., Brigle, K., Taylor, S. M. & Moran, R. G. Mutations in the reduced folate carrier gene which confer dominant resistance to 5,10-dideazatetrahydrofolate.J. Biol. Chem.273, 25953–25960 (1998).
Gifford, A. J. et al. Role of the E45K-reduced folate carrier gene mutation in methotrexate resistance in human leukemia cells.Leukemia16, 2379–2387 (2002).
Zhao, R., Assaraf, Y. G. & Goldman, I. D. A mutated murine reduced folate carrier (RFC1) with increased affinity for folic acid, decreased affinity for methotrexate, and an obligatory anion requirement for transport function.J. Biol. Chem.273, 19065–19071 (1998).
Jansen, G. et al. A structurally altered human reduced folate carrier with increased folic acid transport mediates a novel mechanism of antifolate resistance.J. Biol. Chem.273, 30189–30198 (1998).
Drori, S., Jansen, G., Mauritz, R., Peters, G. J. & Assaraf, Y. G. Clustering of mutations in the first transmembrane domain of the human reduced folate carrier in GW1843U89-resistant leukemia cells with impaired antifolate transport and augmented folate uptake.J. Biol. Chem.275, 30855–30863 (2000).
Zhao, R., Assaraf, Y. G. & Goldman, I. D. A reduced folate carrier mutation produces substrate-dependent alterations in carrier mobility in murine leukemia cells and methotrexate resistance with conservation of growth in 5-formyltetrahydrofolate.J. Biol. Chem.2737873 - 7879 (1998).
Rosowsky, A., Wright, J. E., Vaidya, C. M. & Forsch, R. A. The effect of side-chain,para-aminobenzoyl region, and B-ring modifications on dihydrofolate reductase binding, influx via the reduced folate carrier, and cytotoxicity of the potent nonpolyglutamatable antifolate Nα-(4-amino-4-deoxypteroyl)-Nδ-hemiphthaloyl-L-ornithine.Pharmacol. Ther.85, 191–205 (2000).
Rhee, M. S., Galivan, J., Wright, J. E. & Rosowsky, A. Biochemical studies on PT523, a potent nonpolyglutamatable antifolate, in cultured cells.Mol. Pharmacol.45, 783–791 (1994).
Furst, D. E. The rational use of methotrexate in rheumatoid arthritis and other rheumatic diseases.Br. J. Rheumatol.36, 1196–1204 (1997).
Schroder, O. & Stein, J. Low dose methotrexate in inflammatory bowel disease: current status and future directions.Am. J. Gastroenterol.98, 530–537 (2003).
侯,z . et al。上皮ovaria的双重目标n cancer via folate receptor alpha and the proton-coupled folate transporter with 6-substituted pyrrolo[2,3-d]pyrimidine antifolates.Mol. Cancer Ther.16, 819–830 (2017).
Aluri, S. et al. Substitutions that lock and unlock the proton-coupled folate transporter (PCFT-SLC46A1) in an inward-open conformation.J. Biol. Chem.294, 7245–7258 (2019).
Desmoulin, S. K. et al. Targeting the proton-coupled folate transporter for selective delivery of 6-substituted pyrrolo[2,3-d]pyrimidine antifolate inhibitors of de novo purine biosynthesis in the chemotherapy of solid tumors.Mol. Pharmacol.78, 577–587 (2010).
Drew, D., North, R. A., Nagarathinam, K. & Tanabe, M. Structures and general transport mechanisms by the major facilitator superfamily (MFS).Chem. Rev.121, 5289–5335 (2021).
Jurrus, E. et al. Improvements to the APBS biomolecular solvation software suite.Protein Sci.27, 112–128 (2018).
Ashkenazy, H. et al. ConSurf 2016: an improved methodology to estimate and visualize evolutionary conservation in macromolecules.Nucleic Acids Res.44, W344–W350 (2016).
Harris, M., Firsov, D., Vuagniaux, G., Stutts, M. J. & Rossier, B. C. A novel neutrophil elastase inhibitor prevents elastase activation and surface cleavage of the epithelial sodium channel expressed inXenopus laevisoocytes.J. Biol. Chem.282, 58–64 (2007).
Di Francesco, V., Di Francesco, M., Decuzzi, P., Palomba, R. & Ferreira, M. Synthesis of two methotrexate prodrugs for optimizing drug loading into liposomes.Pharmaceutics13, 332 (2021).
Goehring, A. et al. Screening and large-scale expression of membrane proteins in mammalian cells for structural studies.Nat. Protoc.9, 2574–2585 (2014).
Shinkarev, V. P., Crofts, A. R. & Wraight, C. A. Spectral analysis of thebc1complex components in situ: beyond the traditional difference approach.Biochim. Biophys. Acta1757, 67–77 (2006).
Zheng, S. Q. et al. MotionCor2: anisotropic correction of beam-induced motion for improved cryo-electron microscopy.Nat. Methods14, 331–332 (2017).
Punjani, A., Rubinstein, J. L., Fleet, D. J. & Brubaker, M. A. cryoSPARC: algorithms for rapid unsupervised cryo-EM structure determination.Nat. Methods14, 290–296 (2017).
Rohou, A. & Grigorieff, N. CTFFIND4: fast and accurate defocus estimation from electron micrographs.J. Struct. Biol.192, 216–221 (2015).
Asarnow, D., Palovcak, E., Cheng, Y. UCSF pyem v0.5.Zenodohttps://doi.org/10.5281/zenodo.3576630(2019).
Zivanov, J. et al. New tools for automated high-resolution cryo-EM structure determination in RELION-3.Elife7, e42166 (2018).
Emsley, P. & Cowtan, K. Coot: model-building tools for molecular graphics.Acta Crystallogr. D Biol. Crystallogr.60, 2126–2132 (2004).
Adams, P. D. et al. PHENIX: a comprehensive Python-based system for macromolecular structure solution.Acta Crystallogr. D Biol. Crystallogr.66, 213–221 (2010).
Chen, V. B. et al. MolProbity: all-atom structure validation for macromolecular crystallography.Acta Crystallogr. D Biol. Crystallogr.66, 12–21 (2010).
Lee, J. et al. CHARMM-GUI Input Generator for NAMD, Gromacs, Amber, Openmm, and CHARMM/OpenMM simulations using the CHARMM36 Additive Force Field.Biophys. J.110, 641a–641a (2016).
Katoh, K., Rozewicki, J. & Yamada, K. D. MAFFT online service: multiple sequence alignment, interactive sequence choice and visualization.Brief Bioinform.20, 1160–1166 (2019).
Gabler, F. et al. Protein sequence analysis using the MPI Bioinformatics Toolkit.Curr. Protoc. Bioinformatics72, e108 (2020).
Jo, S., Kim, T. & Im, W. Automated builder and database of protein/membrane complexes for molecular dynamics simulations.PLoS ONE2, e880 (2007).
Tian, C. et al. ff19SB: amino-acid-specific protein backbone parameters trained against quantum mechanics energy surfaces in solution.J. Chem. Theory Comput.16, 528–552 (2020).
He, X., Man, V. H., Yang, W., Lee, T. S. & Wang, J. A fast and high-quality charge model for the next generation general AMBER force field.J. Chem. Phys.153, 114502 (2020).
Jorgensen, W. L., Chandrasekhar, J., Madura, J. D., Impey, R. W. & Klein, M. L. Comparison of simple potential functions for simulating liquid water.J. Chem. Phys.79, 926–935 (1983).
Case, D. A. et al.AMBER 2021(Univ. California, 2021).
Gotz, A. W. et al. Routine microsecond molecular dynamics simulations with AMBER on GPUs. 1. Generalized Born.J. Chem. Theory Comput.8, 1542–1555 (2012).
Salomon-Ferrer, R., Gotz, A. W., Poole, D., Le Grand, S. & Walker, R. C. Routine microsecond molecular dynamics simulations with AMBER on GPUs. 2. Explicit solvent particle mesh Ewald.J. Chem. Theory Comput.9, 3878–3888 (2013).
Lee, J. et al. CHARMM-GUI supports the Amber force fields.J. Chem. Phys.153, 035103 (2020).
Hopkins, C. W., Le Grand, S., Walker, R. C. & Roitberg, A. E. Long-time-step molecular dynamics through hydrogen mass repartitioning.J. Chem. Theory Comput.111864 - 1874 (2015).
Gao, Y. et al. CHARMM-GUI supports hydrogen mass repartitioning and different protonation states of phosphates in lipopolysaccharides.J. Chem. Inf. Model.61, 831–839 (2021).
Darden, T., York, D. & Pedersen, L. Particle mesh Ewald - anN.Log(N) method for Ewald sums in large systems.J. Chem. Phys.98, 10089–10092 (1993).
Essmann, U. et al. A smooth particle mesh Ewald method.J. Chem. Phys.103, 8577–8593 (1995).
Roe, D. R. & Cheatham, T. E. 3rd PTRAJ and CPPTRAJ: software for processing and analysis of molecular dynamics trajectory data.J. Chem. Theory Comput.9, 3084–3095 (2013).
Pettersen, E. F. et al. UCSF Chimera-a visualization system for exploratory research and analysis.J. Comput. Chem.25, 1605–1612 (2004).
Humphrey, W., Dalke, A. & Schulten, K. VMD: visual molecular dynamics.J. Mol. Graph.14, 33–38 (1996).
Kaufman, Y., Ifergan, I., Rothem, L., Jansen, G. & Assaraf, Y. G. Coexistence of multiple mechanisms of PT523 resistance in human leukemia cells harboring 3 reduced folate carrier alleles: transcriptional silencing, inactivating mutations, and allele loss.Blood107, 3288–3294 (2006).
Kaufman, Y. et al. Reduced folate carrier mutations are not the mechanism underlying methotrexate resistance in childhood acute lymphoblastic leukemia.癌症100, 773–782 (2004).
罗伊,K Tolner, B。,焦立中,j . h & Sirotnak f·M. A single amino acid difference within the folate transporter encoded by the murine RFC-1 gene selectively alters its interaction with folate analogues. Implications for intrinsic antifolate resistance and directional orientation of the transporter within the plasma membrane of tumor cells.J. Biol. Chem.273, 2526–2531 (1998).
Acknowledgements
Cryo-EM data were screened and collected at the Duke University Shared Materials Instrumentation Facility (SMIF) and at the Pacific Northwest Center for Cryo-EM (PNCC) at Oregon Health & Science University (OHSU). We thank N. Bhattacharya at SMIF and J. Myers at the PNCC for assistance with microscope operation. This research was supported by National Institutes of Health grant R01GM137421 (S.-Y.L. and J.H.), American Heart Association fellowship 20PRE35210058 (N.J.W.) and National Science Foundation grant MCB-2111728 (W.I.). A portion of this research was supported by National Institutes of Health grant U24GM129547, performed at the PNCC at OHSU and accessed through EMSL (grid.436923.9), a Department of Energy Office of Science User Facility sponsored by the Office of Biological and Environmental Research. The Duke University SMIF is affiliated with the North Carolina Research Triangle Nanotechnology Network, which is in part supported by the National Science Foundation (ECCS-2025064).
Author information
Authors and Affiliations
Contributions
J.G.F.进行生化制备,样品freezing, grid screening and surface accessibility analysis; N.J.W. performed single-particle 3D reconstruction as well as radiotracer uptake assays; and Y.S. and J.G.F. collected data and J.Y. performed initial biochemical characterization, all under the guidance of S.-Y.L. N.J.W. and S.-Y.L. performed model building and refinement. H.Z. carried out all MD simulations under the guidance of W.I. P.J. synthesized NHS–MTX under the guidance of J.H. N.J.W., J.G.F. and S.-Y.L. wrote the manuscript.
Corresponding author
Ethics declarations
Competing interests
The authors declare no competing interests.
Peer review
Peer review information
Naturethanks Larry Matherly and the other, anonymous, reviewer(s) for their contribution to the peer review of this work.
Additional information
Publisher’s note施普林格自然remains neutral with regard to jurisdictional claims in published maps and institutional affiliations.
扩展数据数据和表
Extended Data Fig. 1 Protein biochemistry, NHS-MTX protein modification and cryo-EM analysis of hRFCEM.
a, Topology diagram of hRFCEMused for structural elucidation.b, Representative gel-filtration profile for the final purification step and representative SDS-PAGE analysis (Coomassie stained) of purified protein used for cryo-EM grid preparation. Protein laddering during SDS-PAGE is common for small membrane proteins, with degree of non-specific oligomerization denoted by the number of asterisks (* monomer, ** dimer, *** trimer, **** tetramer). This purification is performed routinely with very similar results, reliably yielding pure and biochemically stable protein sample.c, Characterization of MTX modification of RFC by NHS-MTX. A representative spectral deconvolution of the MTX-RFC UV-vis spectrum into MTX and pure unlabeled RFC yields a labelling ratio of 1.1:1 MTX:RFC (graph prepared in Prism 8).d, Cryo-EM micrograph for hRFCEMsample (of representative quality for all collected cryo-EM data reported in this study) and 2D-classes of hRFCEM.
Extended Data Fig. 2 Cryo-EM data processing of hRFCEM.
a, Processing workflow for hRFCEM.b, Phenix and cryoSPARC reported Fourier shell correlations, and particle angular distribution for the final focused map.c, Local resolution analysis.d, Cryo-EM density corresponding to hRFCEMTM1-12 (map threshold = 0.20).
Extended Data Fig. 3 Cryo-EM data processing of Apo hRFCEM.
a, Processing workflow for Apo hRFCEM.b, Phenix and cryoSPARC reported Fourier shell correlations, and particle angular distribution for the final map.c, Local resolution analysis.d, Cryo-EM density corresponding to Apo hRFCEMTM1-12 (map阈值= 0.25)。e, Structural superposition of the final refined coordinates for apo hRFCEMand hRFCEMf, Overlay of the final cryo-EM reconstructions (sharpened maps) for apo hRFCEMand hRFCEM(final coordinates for apo hRFCEMshown for reference, hRFCEMmap resampled relative to the Apo hRFCEMmap, with map threshold shown at 0.3).g, Weak, spurious cryo-EM density in the transporter central cavity present in both apo hRFCEMand hRFCEMsharpened maps (Map threshold shown at 0.15 for apo hRFCEM, 0.10 for hRFCEM).
Extended Data Fig. 4 Cryo-EM data processing of hRFCEM-MTX.
a, Processing workflow for MTX modified hRFCEMb, Fourier shell correlation and particle angular distribution for the final reconstructionc, Local resolution analysis of the final reconstruction at two different map thresholdsd, Cryo-EM density corresponding to hRFCEM-MTX TM1–12 (map threshold = 0.2).e, Structural superposition of the final refined coordinates for apo hRFCEM(grey) and hRFCEM-MTX (blue), highlighting apparent ligand induced changes in the TM4 conformational statef, Cryo-EM density corresponding to R133 in the apo hRFCEMand hRFCEM-MTX reconstructions (hRFCEMmap resampled relative to the hRFCEM-MTX map, with map threshold shown at 0.1)g, Ribbon depiction of superposed structures.
Extended Data Fig. 5 Human disease and drug resistance associated mutations in hRFC.
Mapping of clinically relevant mutations of full-length RFC11,35,36,37,38,39,40,41,42,83,84,85onto the hRFCEM-MTX structure fall into two general regions. MTX-resistance associated mutations observed in tumor samples (red), in cell lines (green), or observed in both tumors and in cell lines (blue). The putative position of the megaloblastic anemia-associated mutation of ΔPhe212 is shown in purple as the model only extends to residue 211. Other residues not resolved in the structure are listed in parenthesis in the legend.
Extended Data Fig. 6 MD simulations of hRFCEMwith MTX and PT523.
a, The all-atom molecular dynamics system setup for MTX-bound hRFC embedded in a POPC membrane and solvated with 150 mM KCl (Extended Data Table3). hRFC is shown in cartoon, the N- and C- terminal domains colored in blue and yellow, respectively. MTX is shown as sticks, in pink. Lipids are depicted as spheres with glycerol-palmitoyl and -oleoyl groups colored gray, phosphates in orange, and choline in green. Red and blue spheres represent Cl−and K+, respectively.b, Timecourse traces forn = 5 replicates for MD simulations of MTX-K411 hRFC (MD system “MTX-LYS”).c, Timecourse traces forn = 5 replicates for MD simulations of hRFC with unlinked MTX (MD system “MTX”). For b and c, distances from the MTX N4 to E123 carboxylate center-of-mass (blue), and thel-Glu center-of-mass of MTX to Arg guanidiniums (R133, red; R157, purple; R373, cyan), are plotted as a function of time.d, Histogram plot of MTX N4 to E123 distances overn = 5 replicates.e. Histogram plots of MTX α- and γ- carboxylates to Arg guanidiniums overn = 5 replicates.f–h, MD simulations of PT523 docked into hRFC.f, Chemical structure of PT523 compared to MTX with the structural difference highlighted in red.g, the cryo-EM structure of MTX-labelled hRFC versus the MD simulation of docked PT523 (MD system “PT523”), with snapshots taken at 500 ns forn = 5 replicates.h, Snapshots from an MD simulation of docked PT523 sampled at various timepoints.
Extended Data Fig. 7 Evolutionary determinants of ion selectivity within the SLC19 family.
a, Select regions of a multiple sequence alignment of hSLC19A1, hSLC19A2, hSLC19A3. Numbering consistent with sequence position for hSLC19A1b,The mutational effects of R133E and K411Q on the surface electrostatics of hRFCEMby APBS51calculations in PyMOL.c, Ion probability densities from MD simulations of hRFCEMwith and withoutin silicointroduced mutants, becoming gradually more thiamine transporter-2 (hSLC19A3) like. Simulations performed in the presence of 150 mM KCl, with a threshold value of 25 shown for chloride or potassium for each simulation.
Extended Data Fig. 8 Antifolate drug recognition by human RFC and chicken proton-coupled folate transporter (PCFT).
a, Surface representation of the cavities for inward-facing hRFCEM-MTX and outward-facing pemetrexed (PMX)-bound PCFT. The orientation flip of the drugs in the cavities is highlighted by labelling the chemical groups. 0b, Chemical structure and immediate coordinating environment for MTX and PMX, with hydrogen bonds shown as red dashed lines and charged interactions highlighted by denoting charges. α- and γ- carboxylates are highlighted based on their extent of interactions in either system.c, The electrostatic environment of MTX and PMX as calculated by APBS61of the hRFCEM-MTX and PCFT, respectively. Residues frombare shown as sticks with the anion binding site of RFC and selectivity pocket of PCFT as denoted.
Supplementary information
Supplementary Information
This file contains Supplementary Figs. 1 and 2.
Supplementary Video 1
MD simulation of hRFC–MTX. A representative movie of a 1-µs MD simulation of hRFC–MTX, where MTX is covalently linked to K411. Functionally important residues E123, R133, R157 and R373 (green) and K411–MTX (salmon) are depicted as sticks. The protein in the cartoon is coloured by the N-terminal half (blue) and C-terminal half (yellow).
Supplementary Video 2
MD simulation of hRFC with unlinked MTX. A representative movie of a 2-µs MD simulation of hRFC with MTX unlinked from K411. MTX (salmon) and functionally important residues E123, R133, R157 and R373 (green) are shown as sticks. The protein in the cartoon is coloured by its N-terminal half (blue) and C-terminal half (yellow).
Supplementary Video 3
MD simulation of hRFC with PT523. A representative movie of a 0.7-µs MD simulation of hRFC with PT523 docked into the MTX–hRFC structure (with MTX removed). PT523 (salmon) and functionally important residues E123, R133, R157 and R373 (green) are shown as sticks. The protein in the cartoon is coloured by its N-terminal half (blue) and C-terminal half (yellow).
Source data
Rights and permissions
施普林格自然or its licensor (e.g. a society or other partner) holds exclusive rights to this article under a publishing agreement with the author(s) or other rightsholder(s); author self-archiving of the accepted manuscript version of this article is solely governed by the terms of such publishing agreement and applicable law.
About this article
Cite this article
Wright, N.J., Fedor, J.G., Zhang, H.et al.Methotrexate recognition by the human reduced folate carrier SLC19A1.Nature609, 1056–1062 (2022). https://doi.org/10.1038/s41586-022-05168-0
Received:
Accepted:
Published:
Issue Date:
DOI:https://doi.org/10.1038/s41586-022-05168-0
This article is cited by
Folate transporter offers clues for anticancer drugs
Nature(2022)
Recognition of cyclic dinucleotides and folates by human SLC19A1
Nature(2022)
Structural study could aid design of antifolates
Nature Reviews Cancer(2022)
Comments
By submitting a comment you agree to abide by ourTermsandCommunity Guidelines. If you find something abusive or that does not comply with our terms or guidelines please flag it as inappropriate.