Abstract
The Jahn–Teller effect, in which electronic configurations with energetically degenerate orbitals induce lattice distortions to lift this degeneracy, has a key role in many symmetry-lowering crystal deformations1. Lattices of Jahn–Teller ions can induce a cooperative distortion, as exemplified by LaMnO3(refs.2,3). Although many examples occur in octahedrally4or tetrahedrally5coordinated transition metal oxides due to their high orbital degeneracy, this effect has yet to be manifested for square-planar anion coordination, as found in infinite-layer copper6,7, nickel8,9, iron10,11and manganese oxides12. Here we synthesize single-crystal CaCoO2thin films by topotactic reduction of the brownmillerite CaCoO2.5phase. We observe a markedly distorted infinite-layer structure, with ångström-scale displacements of the cations from their high-symmetry positions. This can be understood to originate from the Jahn–Teller degeneracy of thedxzanddyzorbitals in thed7electronic configuration along with substantial ligand–transition metal mixing. A complex pattern of distortions arises in a\(2\sqrt{2}\times 2\sqrt{2}\times 1\)tetragonal supercell, reflecting the competition between an ordered Jahn–Teller effect on the CoO2sublattice and the geometric frustration of the associated displacements of the Ca sublattice, which are strongly coupled in the absence of apical oxygen. As a result of this competition, the CaCoO2structure forms an extended two-in–two-out type of Co distortion following ‘ice rules’13.
This is a preview of subscription content,access via your institution
Access options
Access Nature and 54 other Nature Portfolio journals
Get Nature+, our best-value online-access subscription
$29.99 per month
cancel any time
Subscribe to this journal
Receive 51 print issues and online access
$199.00 per year
only $3.90 per issue
Rent or buy this article
Get just this article for as long as you need it
$39.95
Prices may be subject to local taxes which are calculated during checkout
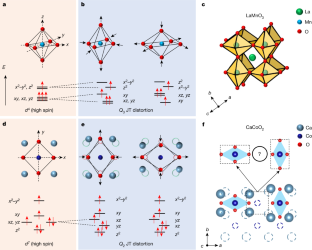
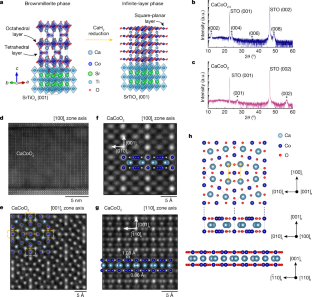
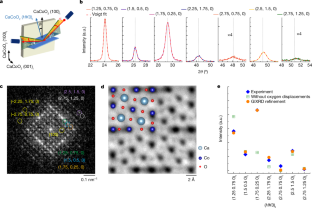
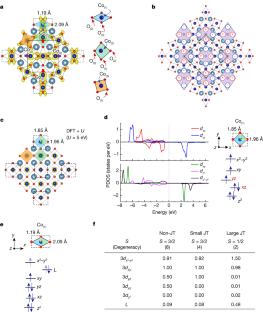
Data availability
The data presented in the figures and other findings of this study are available from the corresponding authors upon reasonable request.
References
Jahn, H. A. & Teller, E. Stability of polyatomic molecules in degenerate electronic states I—orbital degeneracy.Proc. R. Soc. Lond.161, 220–235 (1937).
Goodenough, J. B. Theory of the role of covalence in the perovskite-type manganites [La,M(II)]MnO3.理论物理。Rev.100, 564–573 (1955).
Gehring, G. A. & Gehring, K. A. Co-operative Jahn–Teller effects.Rep. Prog. Phys.38, 1–89 (1975).
Goodenough, J. B. Jahn–Teller phenomena in solids.Annu. Rev. Mater. Sci.28, 1–27 (1998).
Goodenough, J. B. Jahn–Teller distortions induced by tetrahedral-site Fe2+ions.J. Phys. Chem. Solids25, 151–160 (1964).
Siegrist, T., Zahurak, S. M., Murphy, D. W. & Roth, R. S. The parent structure of the layered high-temperature superconductors.Nature334, 231–232 (1988).
Smith, M. G., Manthiram, A., Zhou, J., Goodenough, J. B. & Markert, J. T. Electron-doped superconductivity at 40 K in the infinite-layer compound Sr1–yNdyCuO2.Nature351, 549 - 551(1991)。
Crespin, M., Levitz, P. & Gatineau, L. Reduced forms of LaNiO3perovskite. Part 1.—Evidence for new phases: La2Ni2O5and LaNiO2.J. Chem. Soc. Faraday Trans. 279, 1181–1194 (1983).
Hayward, M. A., Green, M. A., Rosseinsky, M. J. & Sloan, J. Sodium hydride as a powerful reducing agent for topotactic oxide deintercalation: synthesis and characterization of the nickel(I) oxide LaNiO2.J. Am. Chem. Soc.121, 8843–8854 (1999).
Tsujimoto, Y. et al. Infinite-layer iron oxide with a square-planar coordination.Nature450, 1062–1065 (2007).
Kawakami, T. et al. Spin transition in a four-coordinate iron oxide.Nat. Chem.1, 371–376 (2009).
Dixon, E., Hadermann, J., Ramos, S., Goodwin, A. L. & Hayward, M. A. Mn(I) in an extended oxide: the synthesis and characterization of La1–xCaxMnO2+δ(0.6 ≤x≤ 1).J. Am. Chem. Soc.133, 18397–18405 (2011).
Pauling, L. The structure and entropy of ice and of other crystals with some randomness of atomic arrangement.J. Am. Chem. Soc.57, 2680–2684 (1935).
von Helmolt, R., Wecker, J., Holzapfel, B., Schultz, L. & Samwer, K. Giant negative magnetoresistance in perovskitelike La2/3Ba1/3MnOxferromagnetic films.理论物理。Rev. Lett.71, 2331–2333 (1993).
Raveau, B., Hervieu, M., Maignan, A. & Martin, C. The route to CMR manganites: what about charge ordering and phase separation?J. Mater. Chem.11, 29–36 (2001).
汉, J. E., Gunnarsson, O. & Crespi, V. H. Strong superconductivity with local Jahn–Teller phonons in C60solids.理论物理。Rev. Lett.90, 167006 (2003).
Millis, A. J., Littlewood, P. B. & Shraiman, B. I. Double exchange alone does not explain the resistivity of La1–xSrxMnO3.理论物理。Rev. Lett.74, 5144–5147 (1995).
Millis, A. J., Shraiman, B. I. & Mueller, R. Dynamic Jahn–Teller effect and colossal magnetoresistance in La1–xSrxMnO3.理论物理。Rev. Lett.77, 175–178 (1996).
Röder, H., Zang, J. & Bishop, A. R. Lattice effects in the colossal-magnetoresistance manganites.理论物理。Rev. Lett.76, 1356–1359 (1996).
Guzmán-Verri, G. G., Brierley, R. T. & Littlewood, P. B. Cooperative elastic fluctuations provide tuning of the metal–insulator transition.Nature576, 429–432 (2019).
Keller, H., Bussmann-Holder, A. & Müller, K. A. Jahn–Teller physics and high-Tcsuperconductivity.Mater. Today11, 38–46 (2008).
Wurzenberger, X., Piotrowski, H. & Klüfers, P. A stable molecular entity derived from rare iron(II) minerals: the square-planar high-spin-d6FeIIO4chromophore.Angew. Chem. Int. Ed.50, 4974–4978 (2011).
Li, D. et al. Superconductivity in an infinite-layer nickelate.Nature572, 624–627 (2019).
Zeng, S. et al. Phase diagram and superconducting dome of infinite-layer Nd1–xSrxNiO2thin films.理论物理。Rev. Lett.125, 147003 (2020).
Boullay, P. et al. Structure determination of a brownmillerite Ca2Co2O5thin film by precession electron diffraction.理论物理。启B79, 184108 (2009).
Xiang, L. et al. Exceptional oxygen evolution reactivities on CaCoO3and SrCoO3.Sci. Adv.5, eaav6262 (2022).
Wang, Z. L. & Yin, J. S. Cobalt valence and crystal structure of La0.5Sr0.5CoO2.25.Phil. Mag. B77, 49–65 (1998).
Li, H.-B. et al. Dehydration of electrochemically protonated oxide: SrCoO2with square spin tubes.J. Am. Chem. Soc.143, 17517–17525 (2021).
Hayward, M. A. et al. The hydride anion in an extended transition metal oxide array: LaSrCoO3H0.7.Science295, 1882–1884 (2002).
陆:et al。电场控制的三态phase transformation with a selective dual-ion switch.Nature546, 124–128 (2017).
Osada, M. et al. Nickelate superconductivity without rare-earth magnetism: (La,Sr)NiO2.Adv. Mater.33, 2104083 (2021).
Williams, J. H. The molecular electric quadrupole moment and solid-state architecture.Acc. Chem. Res.26, 593–598 (1993).
Radaelli, P. G. et al. Formation of isomorphic Ir3+and Ir4+octamers and spin dimerization in the spinel CuIr2S4.Nature416, 155–158 (2002).
Khomskii, D. I. & Streltsov, S. V. Orbital effects in solids: basics, recent progress, and opportunities.Chem. Rev.121, 2992–3030 (2021).
Chowdhury, S. et al. Negative charge-transfer energy in SrCoO2.5thin films: an interplay between O-2phole density, charge-transfer energy, charge disproportionation, and ferromagnetic ordering.ACS Appl. Electron. Mater.2, 3859–3870 (2020).
Potze, R. H., Sawatzky, G. A. & Abbate, M. Possibility for an intermediate-spin ground state in the charge-transfer material SrCoO3.理论物理。启B51, 11501–11506 (1995).
Cui, B. et al. Direct imaging of structural changes induced by ionic liquid gating leading to engineered three-dimensional meso-structures.Nat. Commun.9, 3055 (2018).
Hidaka, M., Inoue, K., Yamada, I. & Walker, P. J. X-ray diffraction study of the crystal structures of K2CuF4and K2CuxZn1−xF4.Physica B+C121, 343–350 (1983).
Aguado, F., Rodríguez, F., Valiente, R., Señas, A. & Goncharenko, I. Three-dimensional magnetic ordering in the Rb2CuCl4layer perovskite—structural correlations.J. Phys. Condens. Matter16, 1927–1938 (2004).
Cammarata, A. & Rondinelli, J. M. Ferroelectricity from coupled cooperative Jahn–Teller distortions and octahedral rotations in ordered Ruddlesden–Popper manganates.理论物理。启B92, 14102 (2015).
Savitzky, B. H. et al. Image registration of low signal-to-noise cryo-STEM data.Ultramicroscopy191, 56–65 (2018).
Campbell, B. J., Stokes, H. T., Tanner, D. E. & Hatch, D. M. ISODISPLACE: a web-based tool for exploring structural distortions.J. Appl. Crystallogr.39, 607–614 (2006).
Stokes, H. T., Hatch, D. M. & Wells, J. D. Group-theoretical methods for obtaining distortions in crystals: applications to vibrational modes and phase transitions.理论物理。启B43, 11010–11018 (1991).
Perdew, J. P., Burke, K. & Ernzerhof, M. Generalized gradient approximation made simple.理论物理。Rev. Lett.77, 3865–3868 (1996).
Dudarev, S. L., Botton, G. A., Savrasov, S. Y., Humphreys, C. J. & Sutton, A. P. Electron-energy-loss spectra and the structural stability of nickel oxide: an LSDA+Ustudy.理论物理。启B57, 1505–1509 (1998).
Kresse, G. & Hafner, J. Ab initio molecular dynamics for liquid metals.理论物理。启B47, 558–561 (1993).
Ruzsinszky太阳,J。,a & Perdew j.p.强烈constrained and appropriately normed semilocal density functional.理论物理。Rev. Lett.115, 036402 (2015).
Tassel, C. et al. CaFeO2: a new type of layered structure with iron in a distorted square planar coordination.J. Am. Chem. Soc.131, 221–229 (2009).
Goodge, B. H. et al. Doping evolution of the Mott–Hubbard landscape in infinite-layer nickelates.Proc. Natl Acad. Sci. USA118, e2007683118 (2021).
Momma, K. & Izumi, F. VESTA: a three-dimensional visualization system for electronic and structural analysis.J. Appl. Crystallogr.41, 653–658 (2008).
Acknowledgements
We thank W.-S. Lee for discussions. The work at SLAC and Stanford was supported by the US Department of Energy (DOE), Office of Basic Energy Sciences, Division of Materials Sciences and Engineering (contract number DE-AC02-76SF00515) and the Gordon and Betty Moore Foundation’s Emergent Phenomena in Quantum Systems Initiative (grant number GBMF9072, synthesis equipment and initial development). Electron microscopy at Cornell was support by the Department of Defense Air Force Office of Scientific Research (number FA 9550-16-1-0305) and the Packard Foundation, and made use of the Cornell Center for Materials Research Shared Facilities which are supported through the NSF MRSEC programme (DMR-1719875), with the Thermo Fisher Helios G4 UX focused ion beam also supported by NSF (DMR-1539918). The Thermo Fisher Spectra 300 X-CFEG was acquired with support from PARADIM, an NSF MIP (DMR-2039380), and Cornell University. M.A.S. acknowledges additional support from the NSF GRFP under award number DGE-1650441. The 3A beamline at PLS-II is supported in part by MSIT. B.-G.C. is currently affiliated to Korea Research Institute of Standards and Science (KRISS). D.J. acknowledges funding by the Alexander-von-Humboldt foundation via a Feodor Lynen postdoctoral fellowship. Raman spectroscopy measurement was performed at the Stanford Nano Shared Facilities (SNSF), supported by the National Science Foundation under award ECCS-2026822. TOF-SIMS characterization was conducted at the Center for Nanophase Materials Sciences, which is a DOE Office of Science User Facility, and using instrumentation within ORNL’s Materials Characterization Core provided by UT-Battelle, LLC under contract number DE-AC05-00OR22725. The computational work for this project was performed on the Sherlock cluster in the Stanford Research Computing Center.
Author information
Authors and Affiliations
Contributions
W.J.K. and H.Y.H. conceived and designed the experiments. M.A.S., B.H.G. and L.F.K. performed the STEM and EELS measurements and analysis. C.J. performed the DFT calculations. C.J., B.M. and T.P.D. performed the cluster calculations. D.J. performed Raman spectroscopy measurements. W.J.K. grew the samples, which were characterized by W.J.K., K.L., D.J. and M.O. W.J.K. and B.-G.C. performed and analysed the synchrotron GIXRD measurements. A.V.I. performed TOF-SIMS measurements. W.J.K., T.P.D. and H.Y.H. wrote the manuscript, with input from all authors.
Corresponding authors
Ethics declarations
Competing interests
The authors declare no competing interests.
Peer review
Peer review information
Naturethanks Eric Hoglund and the other, anonymous, reviewer(s) for their contribution to the peer review of this work.Peer reviewer reportsare available.
Additional information
Publisher’s noteSpringer Nature remains neutral with regard to jurisdictional claims in published maps and institutional affiliations.
Extended data figures and tables
Extended Data Fig. 1 Structural characterizations for CaCoO2.5and CaCoO2.
a, Atomic-resolution HAADF-STEM image along the [100]tzone-axis projection of CaCoO2.5showing alternate stacking of tetrahedral and octahedral layers.b, X-ray diffraction reciprocal space map of CaCoO2around the (−103) SrTiO3diffraction peak, indicating that the film is relaxed from the substrate.c, Empirical relationship between perovskite and infinite-layer lattice parameters.c-axis lattice parameters for various transition metal oxide compounds are plotted for the perovskite phase and the infinite-layer phase after topotactic reduction. The dashed line is a linear fit for all the data points in the plot. Note that the CaFeO2有相对较大的cinfinite layer/cperovskiteassociate with out-of-plane displacement of both FeO4and Ca layers48.
Extended Data Fig. 2 EELS measurements of CaCoO2.
a, Co-L3,2edge; the blue (red) solid line indicates EEL spectra for CaCoO2.5(CaCoO2).b, Ca-L3,2edge EELS shows that there are no substantial changes in the spectra before (CaCoO2.5, blue) and after reduction (CaCoO2, red).c, A plot of the intensity ratioI(L3)/I(L2) of the Co-L3,2edge for different Co compounds with different oxidation states. Note that the dashed line indicates a polynomial fit curve for four different compounds from ref.27(CoCO3, CoSO4, Co3O4, and CoSi4).I(L3)/I(L2) of the CaCoO2.5and CaCoO2films are depicted with blue and red circles, respectively.d, O K-edges EELS data. Spatially averaged O K-edge spectra of CaCoO2(CaCoO2.5) in red (blue). The partially transparent, solid lines indicate the raw, background-subtracted data, and the dashed lines indicate the Gaussian filtered spectra. Upon reduction of the CaCoO2.5电影CaCoO2, we observe a suppression of the distinct pre-peak at ~ 529 eV in the region of the O K-edge associated with hybridization between O 2pand transition metaldorbitals consistent with a nominal electronic transition from 3d6to 3d7. This is similar to the pre-peak suppression observed upon reduction from perovskite to infinite-layer phase in the related nickelates49. We further see the emergence of a shoulder in the CaCoO2spectrum at ~ 530 eV, which is similar to a feature attributed to ligand hole states in doped infinite-layer nickelates49. This feature is also consistent with published spectra acquired from SrCoO3-δ, which has negative charge transferred state37. An O K-edge spectrum of the SrTiO3substrate is included in black for comparison.
Extended Data Fig. 3 Time-of-flight secondary-ion mass spectrometry (TOF-SIMS) and ABF-STEM measurements of CaCoO2.
a, Depth profiles of H+and other ions from both CaCoO2.5and CaCoO2thin film on SrTiO3substrate (with ~ 2 nm SrTiO3capping layer) were measured with secondary-ion mass spectrometry. The Co ion signals from both CaCoO2.5and CaCoO2thin films were employed as a marker for the interface position. TOF-SIMS measurements show that the H+concentration for CaCoO2is similar to the background level of the as-synthesized CaCoO2.5thin film.b, ABF-STEM image along the [100]tzone-axis projection with overlaid Co, Ca, and O atoms.c, Intensity line profiles for the blue and the orange dashed lines inb. The intensities of the line profiles are from inverted imageb. The blue (orange) solid line indicates the line profile for the Co column (Ca and O column). The peak positions are the relative distances noted at the bottom of the imageb.d, Atomic distances between Ca and Ca (black triangles), Co and Co (green diamonds), Ca and O (red squares), and Ca and Co (blue circles) layers are plotted. Note that the atomic layer numbers indcorrespond to those inb. Error bars are taken as the full-width at half-maximum of the intensity peaks inc.
Extended Data Fig. 4 Powder XRD simulation andc-lattice parameter determination.
Lattice structure models fora, 2\(\sqrt{2}\)at\(\times \)2\(\sqrt{2}\)at\(\times \)ctandb, 2\(\sqrt{2}\)at\(\times \)2\(\sqrt{2}\)at\(\times \)2ct. The second structure model is lattice doubled from the first model by stacking a half-unit-cell shifted layer along the in-plane direction. Powder XRD simulation results for bothc, 2\(\sqrt{2}\)at\(\times \)2\(\sqrt{2}\)at\(\times \)ctandd, 2\(\sqrt{2}\)at\(\times \)2\(\sqrt{2}\)at\(\times \)2ctmodels. Note that the XRD simulation for the 2\(\sqrt{2}\)at\(\times \)2\(\sqrt{2}\)at\(\times \)2ctmodel has a distinct half-order peak along thec-lattice direction. We first founde, the CaCoO2(103)tXRD peak as a reference peak. Based on this reference peak position, we performθ–2θscans along the expected CaCoO2(0.75, 0.25, 0.5) position.f, No XRD peak was observed at the expected CaCoO2(0.75, 0.25, 0.5) peak position, indicating that CaCoO2does not have ac-axis doubling of the simple tetragonal unit cell.
Extended Data Fig. 5 DFT calculations for CaCoO2.
a, Plan-view of the relaxed crystal structure for CaCoO2from DFT + U calculations with U = 2 eV, U = 3 eV, U = 4 eV, U = 5 eV, and U = 6 eV.b, Calculated band dispersion of CaCoO2(DFT + U for U = 5 eV). Green highlightsdxz(anddyz) projections. The inset shows high-symmetry points in the tetragonal Brillouin zone.c, Resistivity versus temperature of CaCoO2thin film. The inset shows that the resistivity is well fitted with an Arrhenius plot with an estimated (transport) gap of 0.337+0.001 eV. The spin-dependent partial density of states (PDOS) ofd, Co(2)ande, Co(3)dorbitals from DFT + U (U = 5 eV). The spin-dependent PDOS of Co(2)shows the degeneracy lifting of thedxz/yx轨道。
Extended Data Fig. 6 Total energy calculation for CaCoO2with\(\sqrt{2}\times \sqrt{2}\times 1\)and\(2\sqrt{2}\times 2\sqrt{2}\times 1\)supercell.
a, DFT+U (U = 5 eV) calculations for the total energy under purely Q2-JT-distortions in the\(\sqrt{2}\times \sqrt{2}\times 1\)supercell.b,\(2\sqrt{2}\times 2\sqrt{2}\times 1\)supercell with different distortion amplitudes. Approaching #10, the structure is approaches the experimentally refined structure.c, Normalized total energy for the structures depicted inb. Three different first-principle calculations are used forc(Methods).
吃晚饭plementary information
Rights and permissions
About this article
Cite this article
Kim, W.J., Smeaton, M.A., Jia, C.et al.Geometric frustration of Jahn–Teller order in the infinite-layer lattice.Nature615, 237–243 (2023). https://doi.org/10.1038/s41586-022-05681-2
Received:
Accepted:
Published:
Issue Date:
DOI:https://doi.org/10.1038/s41586-022-05681-2
Comments
By submitting a comment you agree to abide by ourTermsandCommunity Guidelines. If you find something abusive or that does not comply with our terms or guidelines please flag it as inappropriate.